ABSTRACT
Worldwide, COPD is a major cause of morbidity and mortality. The clinical and functional manifestations of COPD result from lung injury occurring through various mechanisms, including oxidative stress, inflammation, protease-antiprotease imbalance and apoptosis. Oxidative stress is central to the pathogenesis of COPD, since it can directly damage lung structures and exacerbate the other mechanisms involved. The cellular and molecular events involved in such lung injury are believed to occur long before the clinical and functional expression of COPD. Although the use of bronchodilators is currently the principal treatment for COPD, bronchodilators have little or no effect on disease progression. A better understanding of the pathogenesis of COPD, together with renewed efforts in basic and clinical research, will allow the diagnosis of COPD at a pre-clinical stage and provide more appropriate monitoring of disease activity, as well as leading to the development of novel therapeutic agents that will effectively prevent the progression of the disease.
Keywords:
Pulmonary disease, chronic obstructive; Oxidative stress; Oxidants; Antioxidants; Inflammation.
RESUMO
A DPOC é uma causa importante de morbidade e mortalidade em escala global. As manifestações clínicas e funcionais da DPOC resultam de danos pulmonares provocados por um conjunto de mecanismos, incluindo o estresse oxidativo, a inflamação, o desequilíbrio do sistema protease-antiprotease e a apoptose. O estresse oxidativo é central na gênese da DPOC, pois além de provocar dano direto às estruturas pulmonares, amplifica os demais mecanismos. Os eventos celulares e moleculares responsáveis pelo dano pulmonar antecedem em muito a expressão clínica e funcional da DPOC. Os broncodilatadores, principais drogas empregadas atualmente no tratamento da DPOC, não são eficazes em reduzir a progressão da doença. Avanços na compreensão da patogênese da DPOC aliados a esforços renovados na pesquisa básica e clínica deverão permitir sua detecção na fase pré-clínica e possibilitar um monitoramento mais adequado de sua atividade, além de permitir a introdução de novas modalidades de agentes terapêuticos capazes de impedir eficazmente sua progressão.
Palavras-chave:
Doença pulmonar obstrutiva crônica; Estresse oxidativo; Oxidantes; Antioxidantes; Inflamação.
IntroductionWorldwide, COPD is a health problem with severe economic and social repercussions; at the personal level, COPD constitutes a major cause of patient disability and of low quality of life for patients and their caregivers.(1,2) According to the World Health Organization, 80 million people suffer from moderate or severe COPD.(3) In Brazil, the prevalence of COPD in large urban centers such as the city of São Paulo ranges from 6% to over 15%, depending on the diagnostic criteria adopted.(4) The fifth leading cause of death worldwide, COPD will have reached, according to recent estimates, the third position by 2030.(3) The increase in the mortality rate for COPD contrasts with the marked reduction in the mortality rate for diseases such as cancer, coronary disease, cerebrovascular accident and AIDS. This reduction is largely attributed to a greater efficacy in the diagnosis and treatment of these diseases, which in turn results, at least in part, from a better understanding of the etiopathogenic mechanisms of these diseases.
The classical definition of COPD is a chronic and progressive reduction in airflow, secondary to an abnormal inflammatory response of the lungs to the inhalation of noxious particles or toxic gases. This inflammation produces alterations of varying severity in the bronchi (chronic bronchitis), bronchioles (obstructive bronchiolitis), lung parenchyma (emphysema), or any combination of the three.(5,6) In addition to affecting the lungs, COPD is also accompanied by systemic manifestations that have a serious impact on the quality of life and survival of patients, including nutritional depletion and skeletal muscle dysfunction, which contribute to exercise intolerance.(7)
The terms inflammation and airflow reduction are central to the definition of COPD, as well as to that of asthma, although there are marked differences between these two diseases, and those differences should be recognized. The inflammatory cells and mediators observed in the inflammation in COPD are different from those observed in the inflammation in asthma; in addition, the inflammation in COPD does not, in most cases, significantly respond to steroids.(8,9) Furthermore, the reduction in airflow in COPD has a significant and irreversible component, secondary to structural changes in the airways, such as peribronchiolar fibrosis and increased collapsibility, resulting from the destruction of the elastic fibers of the lung tissue. These changes are triggered by a complex mechanism that initiates well before the first clinical and functional manifestations.(10) Therefore, a better understanding of the mechanisms involved in the apparently complex etiopathogenesis of COPD will allow not only an earlier diagnosis but also the development of therapeutic agents that can favorably alter the course of the disease before the development of permanent structural changes.
In general, four principal mechanisms are responsible for the alterations observed in COPD: oxidative stress; inflammation; protease-antiprotease imbalance; and apoptosis.(10) The relative contribution of each of these mechanisms varies and possibly explains the different forms of presentation of the disease. Oxidative stress has been attributed a central role in the pathogenesis of COPD because, in addition to causing direct injury to the respiratory tract, oxidative stress triggers and exacerbates the three other mechanisms mentioned previously.(11-14)
Free radicals and reactive speciesFree radicals are atoms, groups of atoms or molecules that have unpaired electrons on the outer orbital, which explains their instability and high reactivity.(15) However, the term free radical is not ideal to describe the group of reactive pathogenic species, because some of them do not have unpaired electrons on the outer orbital, although they participate in redox reactions. Therefore, the terms reactive oxygen species (ROS) and reactive nitrogen species (RNS) are considered to be more appropriate because they better describe these chemical agents.
The ROS are found in all biological systems and originate from the metabolism of molecular oxygen (O2). Under physiological conditions, O2 undergoes reduction by accepting four electrons, which results in the formation of water.(16) During this process, reactive intermediates such as the superoxide (O2−) radical, the hydrogen peroxide (H2O2) radical and the hydroxyl (OH−) radical are formed (Figure 1). Most of the RNS are formed from the synthesis of nitric oxide (NO) through the conversion of L-arginine into L-citrulline by nitric oxide synthases.(17)
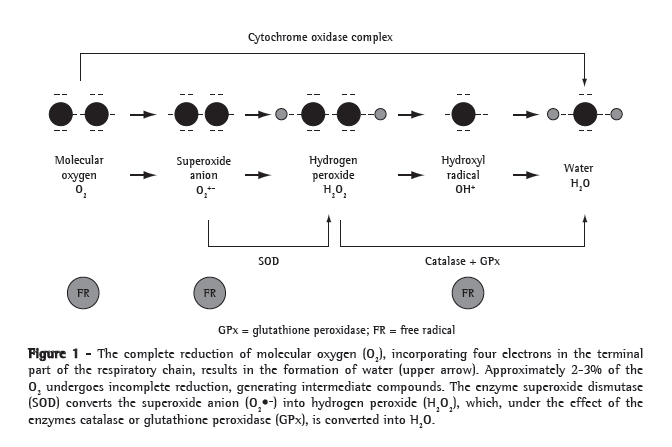
The production of reactive species is an integral part of metabolism and is present under normal conditions, notably in the physiological processes involved in the production of energy, regulation of cell growth, phagocytosis, intracellular signaling and synthesis of important substances, such as hormones and enzymes.(18) In order to offset this production and its potential negative effects, the body has an antioxidant system. In situations in which there is an imbalance between the pro-oxidant system and the antioxidant system (and oxidants predominate), oxidative stress occurs.(18)
Endogenous and exogenous
sources of reactive speciesDue to the fact that the respiratory tract is in direct contact with the external environment and exposed to high concentrations of oxygen, it is a major target of injury caused by oxidants of endogenous or exogenous origin.(18,19)
The reactive species of endogenous origin are generally produced through enzymatic and non-enzymatic reactions of electron transfer. The principal cellular sites and processes that generate oxidants are the mitochondria, the microsomes, the xanthine/xanthine oxidase system and, on a larger scale, NADPH oxidase.(19,20) In the respiratory tract, the principal endogenous sources of oxidants are alveolar macrophages, epithelial cells, endothelial cells and recruited inflammatory cells such as neutrophils, eosinophils, monocytes and lymphocytes.(18) The activation of these cells results in the formation of O2−, which is rapidly converted into H2O2 by the enzyme superoxide dismutase (SOD). Through a non-enzymatic secondary reaction, H2O2 forms, in the presence of iron, OH− (Fenton reaction).(19) The reactive species produced by phagocytes are the principal cause of the tissue injury associated with chronic inflammatory lung diseases.(18) The exogenous oxidants originate from air pollutants such as ozone, nitric dioxide, sulfur dioxide and, in particular, cigarette smoke. Cigarette smoke contains approximately five thousand toxic compounds, including potent oxidants (approximately 1014 free radicals per inhalation) such as acrolein, H2O2, OH− and organic free radicals.(18)
AntioxidantsAccording to their structure, antioxidants can be classified as enzymatic or non-enzymatic. The principal components of the enzymatic antioxidant system are SOD, catalase and glutathione peroxidase (GPx), which are active at the beginning of the reaction through which reactive species are formed, and this avoids the accumulation of O2− radicals and H2O2 radicals. Non-enzymatic antioxidants include compounds produced in vivo, such as reduced glutathione (GSH), ubiquinone, uric acid and the proteins that transport transition metals (transferrin and ceruloplasmin), and compounds obtained directly from the diet, such as beta-carotene, vitamin C and vitamin E.(21,22)
The principal antioxidants in respiratory tract lining fluid include mucin, GSH, uric acid, proteins (especially albumin) and ascorbic acid.(22) Information regarding the antioxidant defense system in the respiratory epithelium of smokers and COPD patients remains scarce. Chronic smokers present elevated levels of GSH in the bronchoalveolar lavage fluid. However, this increase in GSH levels might not be enough to neutralize the excessive load of oxidants during the acute phase of smoking, since a reduction in GSH levels is observed during cigarette smoke exposure, in a time-dependent and dose-dependent manner.(14)
Melatonin, the principal product of the pineal gland, also has significant antioxidant activity and is noteworthy due to some of its characteristics.(23) It is known that melatonin removes reactive species, including singlet O2, O2−, H2O2, OH and lipid hydroperoxide,(23) and, in some cases, melatonin has proved more effective than GSH and vitamin E.(24) The antioxidant effect of melatonin seems to be particularly relevant due to the ability of melatonin to cross all morphophysiological barriers and spread throughout tissues, cells and subcellular compartments. The subcellular distribution of melatonin allows it to interact with toxic molecules in the entire cell, reducing the oxidative damage in the lipid and aqueous media.(23) Melatonin also acts as an indirect antioxidant, through an increase in the activity of the principal antioxidant enzymes, including SOD, catalase and GPx.(23) Unlike other antioxidants, melatonin does not participate in the redox cycle, which allows a molecule to undergo oxidation and reduction repeatedly. The redox cycle allows an antioxidant, such as vitamin C, to act as a pro-oxidant and therefore promote the formation of free radicals. Once oxidized, melatonin cannot be reduced to its former state because it forms stable end products. Due to this characteristic, melatonin is sometimes referred to as a terminal or suicidal antioxidant.
Oxidative stress and etiopathogenic mechanisms of COPDCigarette smoke is the principal environmental factor for the development of COPD. However, the rate of decline in pulmonary function in smokers varies,(25) and only approximately 25% will develop a clinical expression of the disease,(26) suggesting that the pathogenesis of COPD depends on the interaction between environmental and genetic factors.
Emphysema due to alpha-1 antitrypsin deficiency is the only form of expression of COPD that is associated with alterations in a single gene and that does not require interaction with environmental factors. All the other cases of the disease seem to result from molecular alterations that are due to a complex interaction between multiple genes and environmental factors. The genes associated with the alterations observed in COPD include those related to the protease-antiprotease system, antioxidants, inflammation and apoptosis.
Oxidative stress plays an important role in the pathogenesis of COPD through direct injury to the respiratory tract, as well as through exacerbation of the other mechanisms involved (Figure 2).
Oxidative stress and lipid peroxidationLipid peroxidation basically consists of the incorporation of molecular oxygen into a polyunsaturated fatty acid, resulting in oxidative degradation of the latter. Cell membrane phospholipids are particularly susceptible to peroxidation. This leads to alterations in the structure and permeability of the membrane, resulting in loss of ion-exchange selectivity, release of the contents of organelles, such as the hydrolytic enzymes of the lysosomes, and formation of cytotoxic products, such as malondialdehyde (MDA).(27)
In biological systems, cell membrane phospholipids can be hydrolyzed by the phospholipase enzyme, producing nonesterified arachidonic acid, which can undergo peroxidation through two pathways: the enzymatic pathway, involving cyclooxygenases and lipoxygenases, and the non-enzymatic pathway, through the participation of ROS, RNS, transition metals and other free radicals (Figure 3).(27) The end products of the lipid peroxidation mediated by reactive species include 4-hydroxynonenal (4-HNE), MDA and isoprostanes. One isoprostane that has been extensively studied as a marker of pulmonary and systemic oxidative stress is 8-iso-prostaglandin F2α (8-isoprostane).(28)
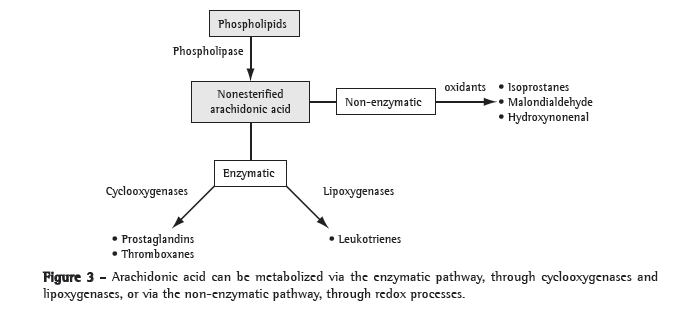
In addition to their importance as markers of oxidative stress, isoprostanes, particularly 8-isoprostane, have various biological effects and are considered to be mediators of oxidative damage.(28) Among the various biological activities of 8-isoprostane is the contraction of the smooth muscles of the bronchi, which is why 8-isoprostane has been reported as being one of the mediators of the reversible component of the obstruction observed in most patients with COPD.(29)
Modification of proteins and DNAIn eukaryotic cells, a protein seldom performs its functions in the form in which it was originally transcribed. After leaving the ribosomes, proteins often undergo modifications, known as post-translational modifications, considered essential to their activity. In addition to these physiological modifications, proteins are subject to a range of modifications produced by reactive species under pathological conditions. These oxidative modifications can inactivate the enzymatic functions and cause structural degeneration of the proteins or activate transcription factors and proteolytic systems.
Markers of oxidative stress and of DNA damage are significantly higher in patients with COPD, especially in those whose causative factor is smoking.(30) Patients with COPD present a high incidence of lung neoplasia, and DNA modifications induced by the reactive species might be the link between these two conditions.
Oxidative stress and inflammationSmokers without COPD present a mild inflammatory response that probably represents a defense reaction of the mucosa against the chronic inhalation of irritants. In individuals who develop COPD, there is a marked exacerbation of the inflammatory response, which increases with the progression of the disease.(31,32) The molecular mechanism of this exacerbation remains unknown; however, genetic factors, latent viral infections and prolonged oxidative stress have been reported as being potentially responsible for the exacerbation.(10,33) Chronic inflammation in COPD is associated with an increase in the production of various mediators and proinflammatory proteins, including cytokines, chemokines, inflammatory enzymes, receptors and adhesion molecules, which are regulated by gene transcription factors.(10,33) Among the mediators, those that are chemotactic for inflammatory cells, in particular leukotriene B4 and IL-8, as well as proinflammatory cytokines, such as TNF-α, IL-1β and IL-6, are noteworthy.(33) Growth factors, including TGF-β, which induces fibrosis in the small airways, are also considered important.(33) The most important cellular elements in the inflammation in COPD are epithelial cells, neutrophils, alveolar macrophages, CD8 lymphocytes and, during periods of exacerbation, eosinophils.(10,33)
Cell response to stimuli depends on a complex signaling process. The stimuli of the extracellular medium are transmitted to the intracellular medium through an organized sequence of reactions, part of which are dependent on redox reactions, generally referred to as redox-sensitive signaling.(19,34) Under physiological conditions, the control of redox-sensitive signaling involves a temporary deviation from the redox state toward an increase in the concentration of oxidants. These small oxidative episodes generate low cellular concentrations of ROS when stimulated by substances such as cytokines (IL-1, IL-6, IL-3 and TNF-α), angiotensin II and growth factors.(19) The signals for the elements responsible for the expression of certain genes are normally transmitted to the nucleus by a class of proteins known as transcription factors. This process of signaling transduction results in biological functions such as muscle contraction, gene expression, cell growth and nervous transmission. Therefore, the initiation and correct functioning of various transduction pathways depend on ROS as signaling molecules, which act as a second messenger.(19) Under pathological conditions, however, abnormally high concentrations of ROS in the cells might lead to permanent changes in signaling transduction and gene expression, as observed in chronic inflammatory diseases, including COPD.
Gene expression might be influenced by the enzymes histone acetyltransferases (HATs) and histone deacetylases (HDACs). The acetylation of lysine residues at the N-terminus of these proteins, catalyzed by HATs, removes positive charges and therefore reduces the DNA affinity of these proteins, making chromatin less compact and more accessible to transcription factors and RNA polymerase. Conversely, deacetylation, catalyzed by HDACs, makes chromatin more compact and less accessible (Figure 4). Consequently, in most cases, HATs intensify transcription, whereas HDACs repress it. Intracellular oxidative stress promotes the activation of the encoding genes of various proinflammatory proteins through two mechanisms: first, by activating the enzyme I kappa B kinase, which degrades the inhibitor protein complex known as inhibitor of kappa B, promoting the release of the nuclear factor NF-kappa B; and second, by inactivating the enzyme histone deacetylase 2 (HDAC2), allowing a greater degree of acetylation of the protein by the HAT enzyme, resulting in chromatin decompaction and allowing transcription factors greater access to genes.(19,31,35)
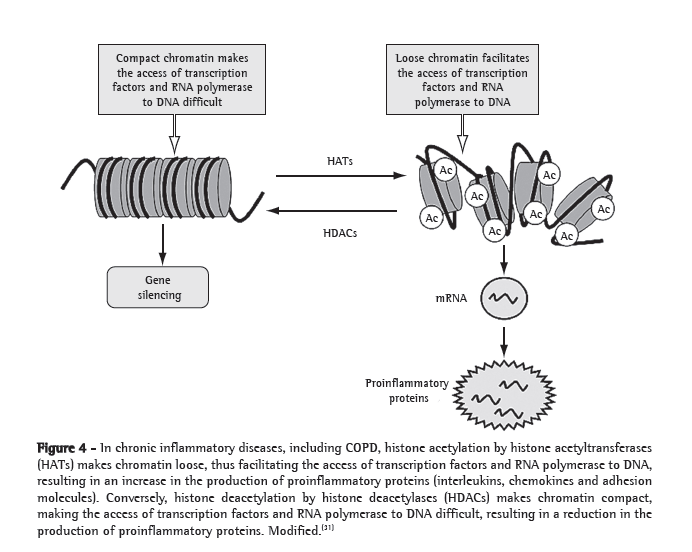
The suppression of proinflammatory genes by glucocorticoids is due, in part, to the recruitment of the enzyme HDAC2, which promotes the compaction of chromatin, thus preventing the transcription of proinflammatory proteins. As mentioned previously, the inflammation in COPD is relatively insensitive to treatment with corticosteroids. One of the mechanisms proposed to explain this relative resistance is the reduction in the levels of the enzyme HDAC2 through oxidative degradation induced by reactive species such as peroxynitrite (ONOO−), acrolein and 4-HNE.(9,35) The alveolar macrophages of patients with COPD present reduced concentrations of HDAC2 when compared with the macrophages of individuals with normal lung function, and this reduction is correlated with insensitivity to corticosteroids. The restoration of the expression of HDAC2 reverses this resistance.(36)
Oxidative stress and protease‑antiprotease imbalanceThere is strong evidence that an imbalance between proteases and endogenous antiproteases plays an important role in the pathogenesis of COPD. Among the factors that contribute to this imbalance are oxidative stress, genetic polymorphism and an abnormal inflammatory response to the respiratory tract injury caused by smoking.(33,37,38)
Three classes of proteases are considered relevant to the etiopathogenesis of COPD: serine proteases, which can degrade elastin and certain forms of collagen; cysteine proteases, which degrade matrix components; and matrix metalloproteinases, which act on collagen, gelatin and laminin. Each of these classes of enzymes can be inhibited by one or more antiproteases.
Oxidants can potentiate the effects of proteases on COPD through the activation of these enzymes. Reactive species increase the activity of matrix metalloproteinases by activating metalloproteinase precursors. The oxidation of methionine residues at active sites of alpha-1 antitrypsin results in a dramatic reduction in their in vitro inhibitory ability; therefore, this pathway has been reported as being one of the possible causes of the imbalance in favor of proteases.(11,33,37,38)
Oxidative stress and apoptosisRecent studies have highlighted the importance of apoptosis in the pathogenesis of COPD.(38,39) It is believed that an increase in the apoptosis of the epithelial and endothelial cells of the lungs, not offset by an increase in the proliferation of these cells, results in tissue destruction and in the development of emphysema.
There is evidence that VEGF is necessary for the maintenance of the cellular structure of the lungs.(40) In fact, VEGF represents a subfamily of growth factors derived from the platelets that bind to three sets of receptors: VEGFR-1, VEGFR-2 and VEGFR-3. The binding of VEGF to VEGFR-2 receptors stimulates endothelial cells and type II pneumocytes, promoting their development and increasing their survival. The interruption of the VEGF signaling to VEGFR-2 receptors results in arrested lung development, which manifests clinically as bronchopulmonary dysplasia in children and as emphysema in adults.(41)
Human emphysema might be associated with a reduced expression of the encoding gene of VEGF. A rat model of emphysema induced by VEGF blockade demonstrated that apoptosis predominates in lung areas under oxidative stress and that the experimental blockade of apoptosis markedly reduced the expression of oxidative stress markers. The administration of compounds with antioxidant activity can prevent the development of alveolar cell apoptosis, suggesting an interaction, through positive feedback, between oxidative stress and apoptosis.(41) It has recently been reported that oxidative stress is associated with a reduction in the levels of VEGF in the sputum of patients with COPD. It has been suggested that oxidative stress can lead to epithelial cell damage, thus reducing the levels of VEGF and, consequently, favoring the development of emphysema.(32) Another mechanism that has been reported as inducing apoptosis through oxidative stress is the activation of certain mitochondrial enzymes, including caspase-3.(39)
Evaluation of the oxidative
stress in COPDOxidative stress can be measured through direct quantification of the production of oxidants or, indirectly, through quantification of the products resulting from lipid peroxidation, such as 8-isoprostane, 4-HNE and MDA, in the alveolar space, in the exhaled air, in the sputum and in the blood.(37)
The collection of exhaled breath condensate (EBC) is a noninvasive method for obtaining samples of material from the lower respiratory tract.(42) This method has been used to determine the concentration of various oxidative stress markers, such as H2O2, NO derivatives (nitrite, nitrate and S-nitrosothiols) and 8-isoprostane, in patients with COPD.(11,42,43)
Various studies showed an increase in oxidative stress markers in the lungs of patients with COPD when compared with normal individuals and with individuals with the same smoking history without COPD.(30,32) Smokers and patients with COPD present higher levels of H2O2 in the EBC (a direct measure of the oxidative load of the air space) than do former smokers with COPD and nonsmokers.(37) Patients with COPD related to the inhalation of smoke from biomass burning present MDA levels similar to those observed in patients with COPD associated with smoking and significantly higher than those in normal controls.(44) During acute exacerbations of COPD, the concentrations of H2O2 are higher than they are during the stable phase of the disease.(37,45) It is believed that high concentrations of H2O2 in the EBC originate, in part, from a greater production of superoxide anions by the alveolar macrophage.(37) The alveolar macrophages of smokers present greater iron content than do those of nonsmokers, resulting in an increase of free iron in the air space, which might stimulate the generation of ROS through the Fenton reaction.(15) An additional source of O2− and H2O2 is the xanthine/xanthine oxidase reaction, which presents increased activity in the cells of the bronchoalveolar lavage fluid and in the plasma of smokers and of patients with COPD when compared with healthy nonsmokers.(15)
The concentration of NO in the gaseous phase of exhaled air has been used as a direct marker of inflammation and, indirectly, of oxidative stress. Patients with COPD present lower levels of NO than do patients with asthma.(46) The rapid reaction between NO and O2−, forming ONOO−, or between NO and thiols, forming nitrosothiols, can change the levels of NO in exhaled air and explain, at least in part, the difference in concentrations between asthma patients and COPD patients. The levels of NO in exhaled air are high in smokers with or without COPD, which limits the validity of this marker in the diagnosis of COPD.
Products of lipid peroxidation, such as thiobarbituric acid reactive substances, are increased in the sputum of patients with COPD and have a negative correlation with FEV1.(11) Through a non-enzymatic reaction, arachidonic acid, undergoing peroxidation mediated by ROS, produces isoprostanes (Figure 3). Patients with COPD present elevated levels of 8-isoprostane in the EBC when compared with normal controls or with smokers without COPD.(47) The levels of 8-isoprostane have a positive correlation with the percentage of neutrophils in induced sputum, which constitutes additional evidence that oxidative stress has a positive modulatory effect on the inflammation in COPD.(43)
Systemic markers of oxidative stress and elevated plasma levels of inflammatory mediators have been reported in smokers and in patients with COPD.(48,49) The peripheral neutrophils of patients with COPD release more ROS than do those of normal nonsmokers.(50) Lipid peroxidation products are also increased in the plasma of smokers with COPD, particularly during periods of exacerbation.(7) Oxidative stress and chronic inflammation are some of the factors involved in the mechanism that generates the systemic manifestations (e.g., weight loss and skeletal muscle dysfunction) observed in some patients with COPD. Patients with COPD are also at increased risk of cardiovascular disease.(7) One of the probable mechanisms for this increase is the endothelial damage caused by systemic inflammation and systemic oxidative stress in these patients.(7)
Treatment and novel
diagnostic methodsThe principal therapeutic agents available for the maintenance treatment of COPD are long-acting bronchodilators, including β2-agonists (formoterol and salmeterol) and anticholinergics (tiotropium), which have no significant impact on the progression of COPD or on COPD-related mortality.
Some factors explain the difficulty in developing more effective drugs for the treatment of COPD. First, animal models of COPD are considered inappropriate for drug trials. Models of emphysema are currently used instead of models of small airway disease; the latter, however, are more appropriate to evaluate potential drugs that can have an early effect on the progression of the disease. Second, studies investigating the effects of drugs on patient-centered outcomes, such as the reduction in loss of pulmonary function and in mortality, are expensive and take longer to be carried out. In addition, various patients with COPD present with comorbidities such as diabetes mellitus and coronary disease, which are commonly among the exclusion criteria for participation in clinical trials of new drugs. Finally, oxidative markers that monitor short-term efficacy, such as those in the blood, sputum and exhaled breath, are still in the validation phase.(42)
More recently, there have been promising reports of novel diagnostic techniques and methods, as well as of potential therapeutic targets that can actually change the clinical course and the prognosis of the disease. Among the novel diagnostic methods, the collection of EBC is noteworthy. It is a simple and noninvasive method that allows us to obtain material from lung tissue in order to detect markers of inflammation and of oxidative stress.(42) In the near future, the determination of the level of oxidative stress in the lungs will probably be used routinely as an indicator of the pathological changes that precede the clinical and functional expression of COPD.
Drugs that act directly or indirectly on the pathogenic mechanisms of COPD, including antagonists to proinflammatory mediators, drugs that inhibit antiproteases and agents that modify the inflammatory response, as well as drugs that can change the resistance of COPD-related inflammation to steroids, are alternatives that can potentially influence the evolution and the prognosis of the disease.(51,52) The therapeutic agents that constitute this last group-modifiers of the effect of corticosteroids on the inflammation in COPD-are considered to be the most promising. As previously mentioned, it is believed that the resistance of COPD-related inflammation to corticosteroids is due to a reduction in the expression and activity of HDAC2 caused by oxidative stress.(31,53,54) It is known that drugs such as xanthines and certain macrolides can increase the expression of HDAC2.(36,55)
In addition to reducing the expression and activity of HDAC2, oxidative stress has been reported to exacerbate other pathogenic mechanisms of COPD.(11,33) Therefore, the use of antioxidant agents potentially reduces the direct damage to lung tissue caused by oxidative stress and the exacerbating effect of oxidative stress on the other mechanisms involved in COPD, as well as reversing the resistance of inflammation to steroids. To date, few antioxidant agents have been tested in the treatment of patients with COPD. It has been shown that N-acetylcysteine, a glutathione precursor, is able to reduce the concentration of H2O2 in the EBC of patients with COPD(56,57); however, it was not able to prevent the deterioration of pulmonary function or to reduce the frequency of exacerbations.(58) N-acetylcysteine is a relatively weak antioxidant, susceptible to inactivation by oxidative stress. It is likely that substances that have a more potent antioxidant effect and are more stable, that is, substances that do not participate in the redox cycle, can lead to a significant decrease in oxidative stress, thus preventing the progression of the disease.
Final considerationsOxidative stress plays an important role in the pathogenesis of COPD, since it can directly damage the components of the respiratory tract and exacerbate the other mechanisms involved. A better understanding of the cellular and molecular events involved in the pathogenesis of COPD will create the basis for new approaches that will allow the diagnosis of COPD at early stages and provide appropriate monitoring of disease activity, as well as leading to the development of novel therapeutic agents to prevent the progression of the disease.
References 1. Pinto RA, Holanda MA, Medeiros MM, Mota RM, Pereira ED. Assessment of the burden of caregiving for patients with chronic obstructive pulmonary disease. Respir Med. 2007;101(11):2402-8.
2. Nunes DM, Mota RM, de Pontes Neto OL, Pereira ED, de Bruin VM, de Bruin PF. Impaired sleep reduces quality of life in chronic obstructive pulmonary disease. Lung. 2009;187(3):159-63.
3. World Health Organization [homepage on the Internet]. Geneva: World Health Organization; c2009 [cited 2009 Jul 2]. World Health Report 2008. Available from: http://www.who.int/whr/2008/en/index.html
4. Menezes AM, Jardim JR, Pérez-Padilla R, Camelier A, Rosa F, Nascimento O, et al. Prevalence of chronic obstructive pulmonary disease and associated factors: the PLATINO Study in São Paulo, Brazil. Cad Saude Publica. 2005;21(5):1565-73.
5. Sociedade Brasileira de Pneumologia e Tisiologia. II Consenso Brasileiro sobre Doença Pulmonar Obstrutiva Crônica DPOC. J Pneumol. 2004;30(Suppl 5):S1 S42.
6. Barnes PJ. Chronic obstructive pulmonary disease. N Engl J Med. 2000;343(4):269-80.
7. Barnes PJ, Celli BR. Systemic manifestations and comorbidities of COPD. Eur Respir J. 2009;33(5):1165-85.
8. Barnes PJ. Mechanisms in COPD: differences from asthma. Chest. 2000;117(2 Suppl):10S-4S.
9. Barnes PJ, Ito K, Adcock IM. Corticosteroid resistance in chronic obstructive pulmonary disease: inactivation of histone deacetylase. Lancet. 2004;363(9410):731-3.
10. MacNee W. Pathogenesis of chronic obstructive pulmonary disease. Proc Am Thorac Soc. 2005;2(4):258-66 ; discussion 290-1.
11. MacNee W. Pulmonary and systemic oxidant/antioxidant imbalance in chronic obstructive pulmonary disease. Proc Am Thorac Soc. 2005;2(1):50-60.
12. MacNee W. Pathogenesis of chronic obstructive pulmonary disease. Clin Chest Med. 2007;28(3):479-513, v.
13. Rahman I, Skwarska E, Henry M, Davis M, O'Connor CM, FitzGerald MX, et al. Systemic and pulmonary oxidative stress in idiopathic pulmonary fibrosis. Free Radic Biol Med. 1999;27(1-2):60-8.
14. MacNee W, Rahman I. Is oxidative stress central to the pathogenesis of chronic obstructive pulmonary disease? Trends Mol Med. 2001;7(2):55-62.
15. Kirkham P, Rahman I. Oxidative stress in asthma and COPD: antioxidants as a therapeutic strategy. Pharmacol Ther. 2006;111(2):476-94.
16. Andrade Jr DR, Souza RB, Santos SA, Andrade DR. Oxygen free radicals and pulmonary disease. J Bras Pneumol. 2005;31(1):60-8
17. Mak JC. Pathogenesis of COPD. Part II. Oxidative-antioxidative imbalance. Int J Tuberc Lung Dis. 2008;12(4):368-74.
18. Rajendrasozhan S, Yang SR, Edirisinghe I, Yao H, Adenuga D, Rahman I.. Deacetylases and NF-kappaB in redox regulation of cigarette smoke-induced lung inflammation: epigenetics in pathogenesis of COPD. Antioxid Redox Signal. 2008;10(4):799-811.
19. Park HS, Kim SR, Lee YC. Impact of oxidative stress on lung diseases. Respirology. 2009;14(1):27-38.
20. Finkel T, Holbrook NJ. Oxidants, oxidative stress and the biology of ageing. Nature. 2000;408(6809):239-47.
21. Vasconcelos SML, Goulart MOF, Moura JBF, Manfredini V, Benfato MS, Kubota LT. Espécies reativas de oxigênio e de nitrogênio, antioxidantes e marcadores de dano oxidativo em sangue humano: principais métodos analíticos para sua determinação. Quim Nova. 2007;30(5):1323-38.
22. MacNee W. Oxidants/antioxidants and COPD. Chest. 2000;117(5 Suppl 1):303S-17S.
23. Tomás-Zapico C, Coto-Montes A. A proposed mechanism to explain the stimulatory effect of melatonin on antioxidative enzymes. J Pineal Res. 2005;39(2):99-104.
24. Sofic E, Rimpapa Z, Kundurovic Z, Sapcanin A, Tahirovic I, Rustembegovic A, et al. Antioxidant capacity of the neurohormone melatonin. J Neural Transm. 2005;112(3):349-58.
25. Fletcher C, Peto R. The natural history of chronic airflow obstruction. Br Med J. 1977;1(6077):1645-8.
26. Løkke A, Lange P, Scharling H, Fabricius P, Vestbo J. Developing COPD: a 25 year follow up study of the general population. Thorax. 2006;61(11):935-9.
27. Lima ES, Abdalla DSP. Peroxidação lipídica: mecanismos e avaliação em amostras biológicas. Rev Bras Cienc Farm. 2001;37(3):293-303.
28. Montuschi P, Barnes PJ, Roberts LJ 2nd. Isoprostanes: markers and mediators of oxidative stress. FASEB J. 2004;18(15):1791-800.
29. Rolin S, Masereel B, Dogné JM. Prostanoids as pharmacological targets in COPD and asthma. Eur J Pharmacol. 2006;533(1-3):89-100.
30. Ceylan E, Kocyigit A, Gencer M, Aksoy N, Selek S. Increased DNA damage in patients with chronic obstructive pulmonary disease who had once smoked or been exposed to biomass. Respir Med. 2006;100(7):1270-6.
31. Barnes PJ. Role of HDAC2 in the Pathophysiology of COPD. Annu Rev Physiol. 2009:71:451-64.
32. Kanazawa H, Yoshikawa J. Elevated oxidative stress and reciprocal reduction of vascular endothelial growth factor levels with severity of COPD. Chest. 2005;128(5):3191-7.
33. Barnes PJ, Shapiro SD, Pauwels RA. Chronic obstructive pulmonary disease: molecular and cellular mechanisms. Eur Respir J. 2003;22(4):672-88.
34. Rajendrasozhan S, Yang SR, Edirisinghe I, Yao H, Adenuga D, Rahman I. Deacetylases and NF-kappaB in redox regulation of cigarette smoke-induced lung inflammation: epigenetics in pathogenesis of COPD. Antioxid Redox Signal. 2008;10(4):799-811.
35. Rahman I, Marwick J, Kirkham P. Redox modulation of chromatin remodeling: impact on histone acetylation and deacetylation, NF-kappaB and pro-inflammatory gene expression. Biochem Pharmacol. 2004;68(6):1255-67.
36. Cosio BG, Tsaprouni L, Ito K, Jazrawi E, Adcock IM, Barnes PJ. Theophylline restores histone deacetylase activity and steroid responses in COPD macrophages. J Exp Med. 2004;200(5):689-95.
37. Owen CA. Proteinases and oxidants as targets in the treatment of chronic obstructive pulmonary disease. Proc Am Thorac Soc. 2005;2(4):373-85; discussion 394-5.
38. Tuder RM, Yoshida T, Arap W, Pasqualini R, Petrache I. State of the art. Cellular and molecular mechanisms of alveolar destruction in emphysema: an evolutionary perspective. Proc Am Thorac Soc. 2006;3(6):503-10.
39. Demedts IK, Demoor T, Bracke KR, Joos GF, Brusselle GG. Role of apoptosis in the pathogenesis of COPD and pulmonary emphysema. Respir Res. 2006;7:53.
40. McGrath-Morrow SA, Cho C, Cho C, Zhen L, Hicklin DJ, Tuder RM. Vascular endothelial growth factor receptor 2 blockade disrupts postnatal lung development. Am J Respir Cell Mol Biol. 2005;32(5):420-7.
41. Tuder RM, Zhen L, Cho CY, Taraseviciene-Stewart L, Kasahara Y, Salvemini D, et al. Oxidative stress and apoptosis interact and cause emphysema due to vascular endothelial growth factor receptor blockade. Am J Respir Cell Mol Biol. 2003;29(1):88-97.
42. Horváth I, Hunt J, Barnes PJ, Alving K, Antczak A, Baraldi E, et al. Exhaled breath condensate: methodological recommendations and unresolved questions. Eur Respir J. 2005;26(3):523-48.
43. Kostikas K, Papatheodorou G, Psathakis K, Panagou P, Loukides S. Oxidative stress in expired breath condensate of patients with COPD. Chest. 2003;124(4):1373-80.
44. Montano M, Cisneros J, Sansores R, Mercado D, Ramirez A, Ramos C. Oxidative Stress in COPD Associated to Biomass Exposure. Am J Respir Crit Care Med. 2009;179:A2952.
45. Gerritsen WB, Asin J, Zanen P, van den Bosch JM, Haas FJ. Markers of inflammation and oxidative stress in exacerbated chronic obstructive pulmonary disease patients. Respir Med. 2005;99(1):84-90.
46. Delen FM, Sippel JM, Osborne ML, Law S, Thukkani N, Holden WE. Increased exhaled nitric oxide in chronic bronchitis: comparison with asthma and COPD. Chest. 2000;117(3):695-701.
47. Montuschi P, Collins JV, Ciabattoni G, Lazzeri N, Corradi M, Kharitonov SA, et al. Exhaled 8-isoprostane as an in vivo biomarker of lung oxidative stress in patients with COPD and healthy smokers. Am J Respir Crit Care Med. 2000;162(3 Pt 1):1175-7.
48. Gan WQ, Man SF, Senthilselvan A, Sin DD. Association between chronic obstructive pulmonary disease and systemic inflammation: a systematic review and a meta-analysis. Thorax. 2004;59(7):574-80.
49. Takabatake N, Nakamura H, Abe S, Inoue S, Hino T, Saito H, et al. The relationship between chronic hypoxemia and activation of the tumor necrosis factor-alpha system in patients with chronic obstructive pulmonary disease. Am J Respir Crit Care Med. 2000;161(4 Pt 1):1179-84.
50. Morrison D, Rahman I, Lannan S, MacNee W. Epithelial permeability, inflammation, and oxidant stress in the air spaces of smokers. Am J Respir Crit Care Med. 1999;159(2):473-9.
51. Barnes PJ. Future treatments for chronic obstructive pulmonary disease and its comorbidities. Proc Am Thorac Soc. 2008;5(8):857-64.
52. de Boer WI, Yao H, Rahman I. Future therapeutic treatment of COPD: struggle between oxidants and cytokines. Int J Chron Obstruct Pulmon Dis. 2007;2(3):205-28.
53. Ito K, Ito M, Elliott WM, Cosio B, Caramori G, Kon OM, et al. Decreased histone deacetylase activity in chronic obstructive pulmonary disease. N Engl J Med. 2005;352(19):1967-76.
54. Ito K, Yamamura S, Essilfie-Quaye S, Cosio B, Ito M, Barnes PJ, et al. Histone deacetylase 2-mediated deacetylation of the glucocorticoid receptor enables NF-kappaB suppression. J Exp Med. 2006;203(1):7-13.
55. Adcock IM, Barnes PJ. Molecular mechanisms of corticosteroid resistance. Chest. 2008;134(2):394-401.
56. Kasielski M, Nowak D. Long-term administration of N-acetylcysteine decreases hydrogen peroxide exhalation in subjects with chronic obstructive pulmonary disease. Respir Med. 2001;95(6):448-56.
57. De Benedetto F, Aceto A, Dragani B, Spacone A, Formisano S, Pela R, et al. Long-term oral n-acetylcysteine reduces exhaled hydrogen peroxide in stable COPD. Pulm Pharmacol Ther. 2005;18(1):41-7.
58. Decramer M, Rutten-van Mölken M, Dekhuijzen PN, Troosters T, van Herwaarden C, Pellegrino R, et al. Effects of N-acetylcysteine on outcomes in chronic obstructive pulmonary disease (Bronchitis Randomized on NAC Cost-Utility Study, BRONCUS): a randomised placebo-controlled trial. Lancet. 2005;365(9470):1552‑60.
Study carried out under the auspices of the Postgraduate Program in Medical Sciences, Department of Clinical Medicine, Federal University of Ceará School of Medicine, Fortaleza, Brazil.
Correspondence to: Pedro Felipe Carvalhedo de Bruin. Departamento de Medicina Clínica, Universidade Federal do Ceará, Rua Prof. Costa Mendes, 1608, 4º andar, CEP 60430-140, Fortaleza, CE, Brasil.
Tel 55 85 3366-8052. E-mail: pfelipe@superig.com.br
Financial support: None.
Submitted: 2 July 2009. Accepted, after review: 18 August 2009.
About the authorsAntonio George de Matos Cavalcante
Physician in the Department of Pulmonology. Walter Cantídio University Hospital, Federal University of Ceará, Fortaleza, Brazil.
Pedro Felipe Carvalhedo de Bruin
Associate Professor. Department of Clinical Medicine, Federal University of Ceará School of Medicine, Fortaleza, Brazil.